I use simulations to study galaxies and how their properties are influenced by their environment. I study galaxies and clusters in the IllustrisTNG simulations and run zoom-in simulations of Mily Way-like halos with self-interacting dark matter models.
Galactic environments and cluster galaxy evolution
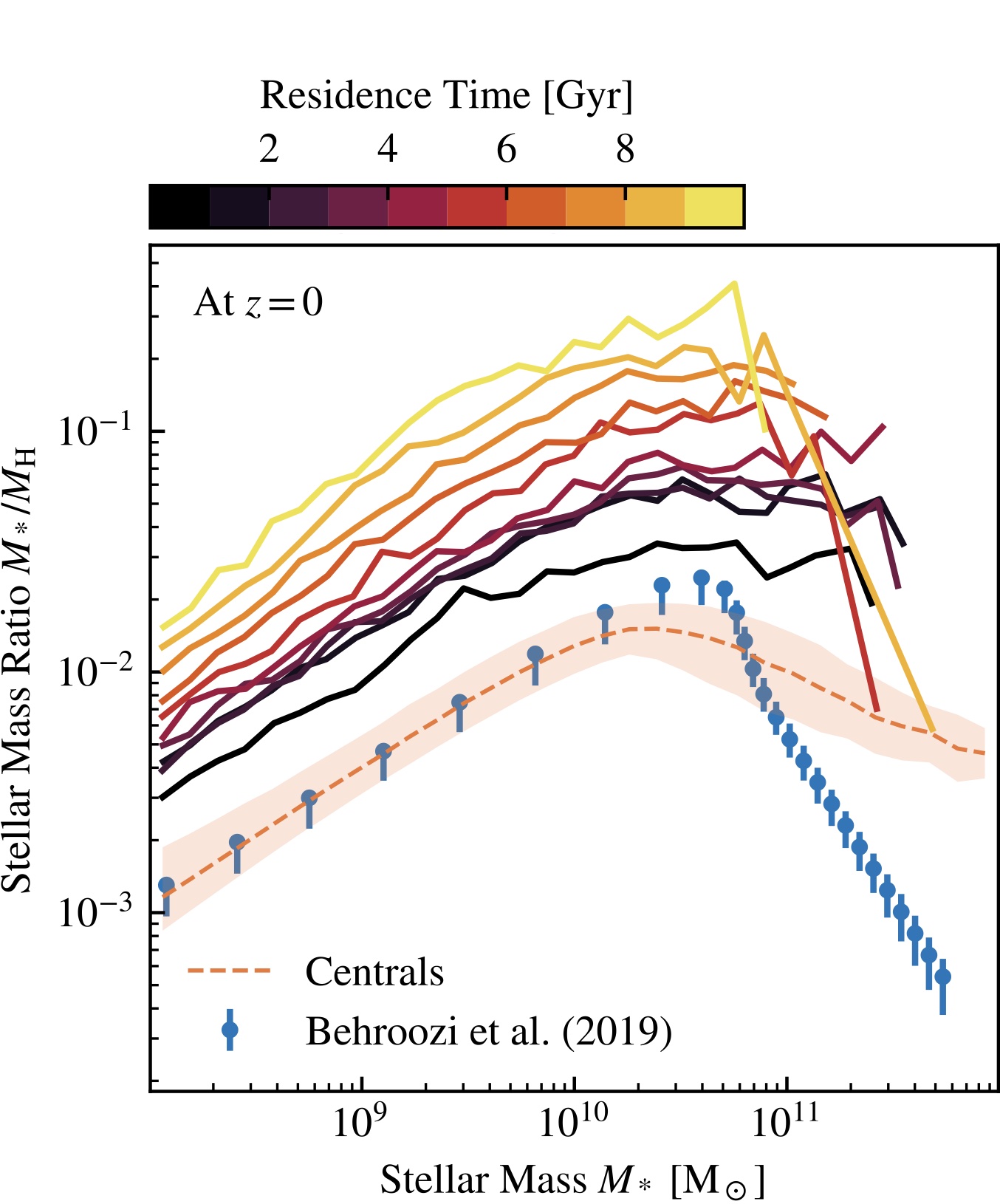
An example of a scaling relation that changes for cluster galaxies compared to field galaxies. The stellar-to-halo mass ratio steadily increases with residence time.
Galaxies come in a vast range of sizes, colors, and morphologies. These are not distributed randomly through the universe, but it is much more likely to find, for example, red elliptical galaxies in galaxy clusters compared to the blue spiral galaxies that are common in the field. A major factor in the difference between cluster and field galaxies is the cluster environment, which is full of hot gas and has a much higher density of galaxies. However, it is not clear what the exact mechanisms are that cause all these changes and how the environment influences galaxy evolution.
I use IllustrisTNG to study how galaxies change as a function of cluster residence time, i.e. how long a galaxy has lived within a cluster. Particularly significant is how this affects commonly used scaling relations. Because we cannot interact directly with galaxies, we rely on calibrated relations between observable quantities like luminosity and quantities of interest like galaxy mass. However, the same relations do not hold for cluster galaxies and field galaxies. I showed that when a galaxy enters a cluster, it continues on an alternative evolutionary pathway compared to field galaxies, and this results in significant and predictable deviations from the standard scaling relations.
Splashback boundary measurements in IllustrisTNG
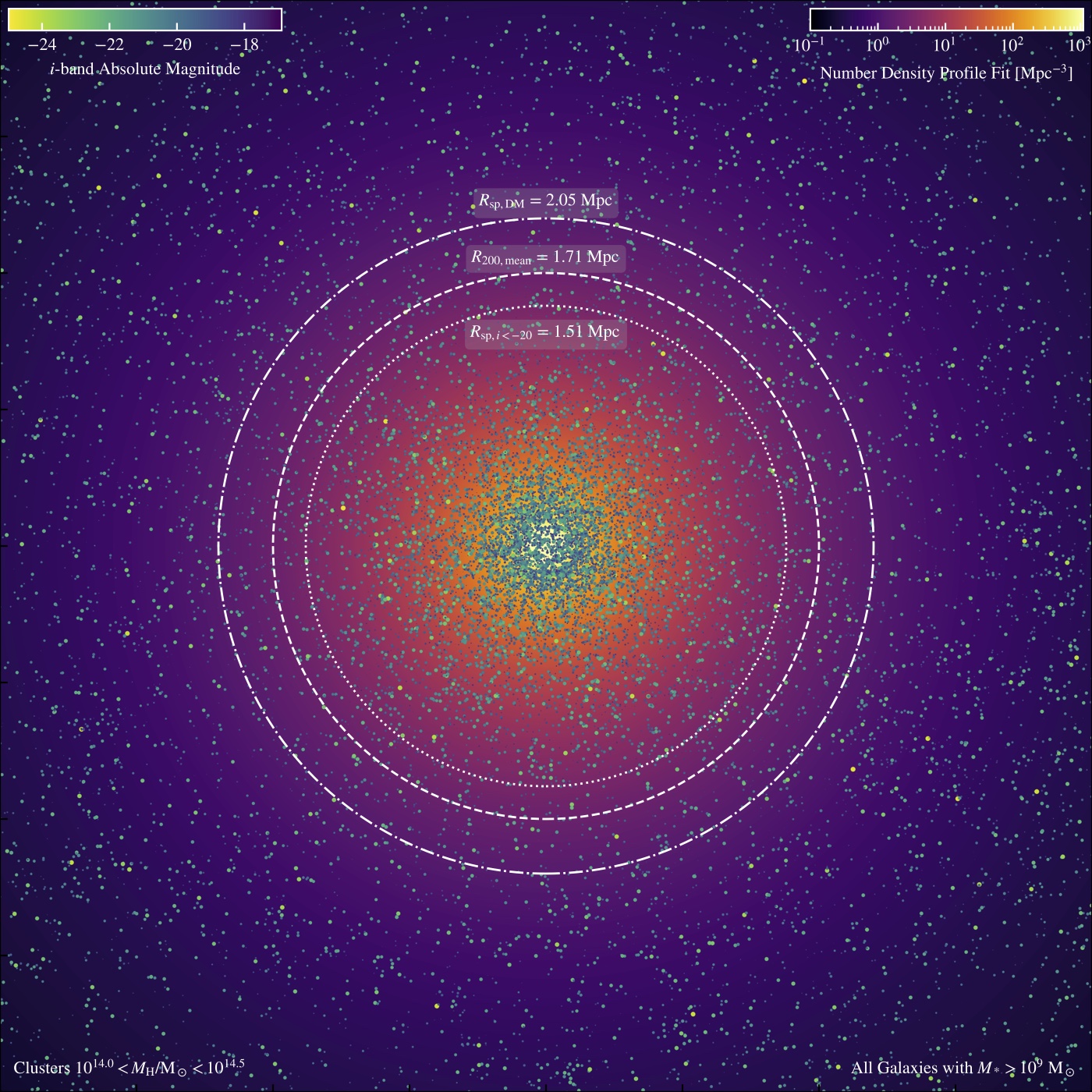
A visual of the dark matter splashback radius, R200,mean, and the splashback radius measured from a population of galaxies. Each point represents a galaxy and the background color corresponds to the density of galaxies.
The size of a dark matter halo is a fundamental property used in a range of studies from cosmology to galaxy evolution to the nature of dark matter. Given the web-like nature of cosmic structure, however, this is not a straightforward quantity to define. The splashback radius describes the boundary of a halo using the orbiting material, specifically the boundary that encloses the first orbits of infalling material. This quantity does not suffer from pseudoevolution like traditional overdensity definitions and reflects the dynamical nature of the halo.
The splashback radius can be measured by identifying the point of steepest slope in the dark matter density profile. A similar feature can be identified observationally using galaxy number density profiles of clusters in galaxy surveys. However, it is unclear how the dark matter measurement and galaxy measurement relate to each other. Cluster galaxies differ from field galaxies since the cluster environment strips them of gas, which quenches their star formation and alters their color. Making splashback measurements with different galaxy populations alters the calculated splashback radius. I use simulations to make splashback measurements with different galaxy populations for the same halos to understand how the measurement changes for different galaxy properties like mass or star formation rate. This allows us to determine the relationship between observational measurements and the underlying dark matter halo and the effect of the galaxy environment on galaxies as well as determine the extent of this environment.
Self-interacting dark matter
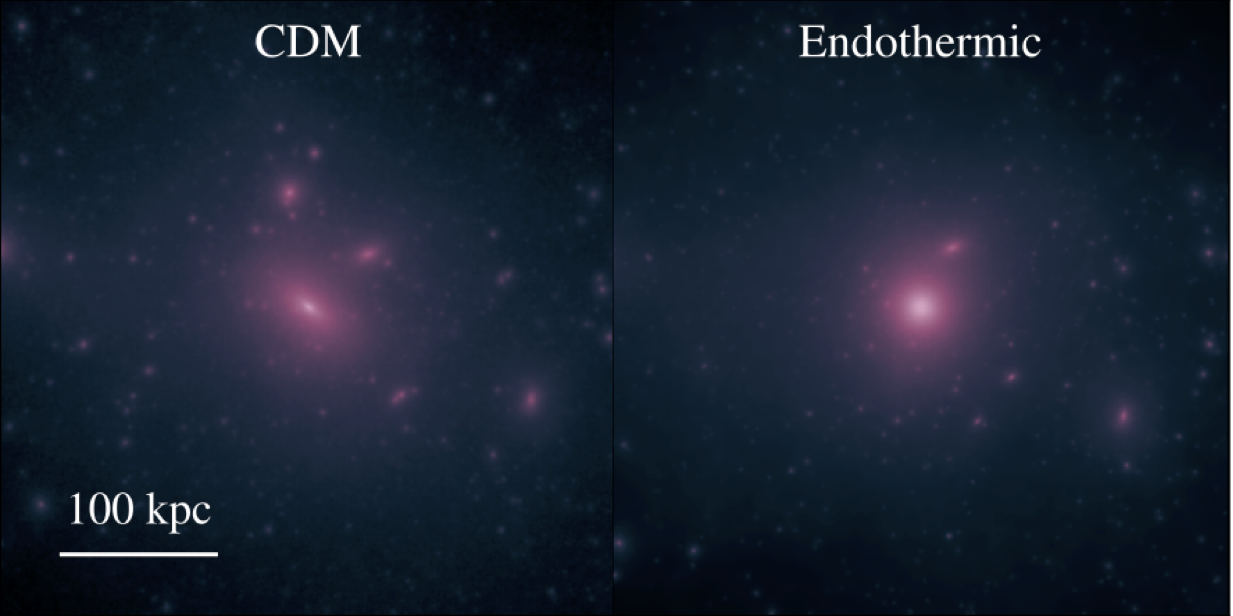
Surface densities of two halos using the same initial conditions but different dark matter models (cold dark matter on the left and self-interacting dark matter on the right).
Roughly 85% of matter and 30% of the total energy budget in the Universe is dark matter. The standard model for dark matter is cold dark matter, in which dark matter particles interact with each other and with baryonic matter only through gravity. While this model reproduces large-scale structure, there are still several discrepencies with small-scale observations. The inner regions of halos, for examples, are more dense in simulations of CDM than observed galaxies. Additionally, we have yet to detect any predicted dark matter particles. Although some of these discrepencies may be resolved through improved modeling of baryonic physics, it may also be that the assumption cold dark matter is incorrect.
I run zoom-in simulations of Milky Way-like halos with alternative dark matter halos. In these simulations, dark matter particles have a ground and excited state. While they still interact with baryons only through gravity, the particles can scatter with each other elastic or inelastically with velocity-dependent cross sections. In elastic scattering, dark matter particles scatter and change their direction of travel without changing energies. This adds a pressure to the dark matter and changes its behavior on small scales. In inelastic scattering, two ground state particles or two excited state particles scatter and change states. The energy difference in changing states turns into kinetic energy, which alters the velocity distribution of dark matter particles which in turn alters the spatial distribution of their orbits. My simulations show how halo properties, like core density or satellite distrubtion, are affected by these interactions.